Menet Lab
Department of Biology, Texas A&M University

Research in the Menet lab focuses on characterizing the mechanisms by which the mammalian circadian clock generates 24-hour rhythms in gene expression. In mammals, more than half of the genome is rhythmically expressed somewhere in the body. These rhythms are initiated by a timekeeping mechanism, the circadian clock, which is present in nearly every cell and drives overt rhythms of most biochemical and physiological processes. The mammalian circadian clock is initiated by the heterodimeric transcription factor CLOCK:BMAL1 (Fig. 1). During the day, CLOCK:BMAL1 binds DNA to activate the transcription of Period (Per1, Per2 and Per3) and Cryptochrome (Cry1 and Cry2), which upon expression form a repressive complex that inhibits CLOCK:BMAL1-mediated transcription at night. Degradation of PERs and CRYs at the end of the night reinitiates a new cycle of rhythmic transcription. CLOCK:BMAL1 also binds to thousands of genomic locations to initiate the rhythmic expression of genes that underlie the daily activity of biological functions.
The importance of rhythmic gene expression is underscored by the wide range of disorders developed by organisms bearing a genetically or environmentally disrupted clock, and which include obesity, diabetes, cardiovascular diseases, and cancer. Defining how rhythmic gene expression is regulated is thus critical for understanding how clock disruption leads to the development of pathologies, and research in our lab is centered around addressing this important question. Our current projects focus more specifically on:
1) how do clock genes rhythmically regulate the chromatin environment?
2) how does rhythmic food intake contribute to driving rhythmic gene expression?

Figure 1: Molecular circadian clockwork in mammals
1. Rhythmic regulation of the chromatin environment by CLOCK:BMAL1
CLOCK:BMAL1 rhythmically binds thousands of sites across the genome, yet, 70% of its target genes are constitutively expressed or not expressed, and peak transcription of some rhythmic targets even occurs at night in antiphase to its daytime peak of DNA binding. Along with some other findings in the field, these results indicate that CLOCK:BMAL1 DNA binding is necessary, but not sufficient, to activate rhythmic transcription. They also imply that CLOCK:BMAL1 uses some uncharacterized regulatory mechanisms to initiate rhythmic transcription.
A major focus of the lab is to identify these mechanisms, as they will shed light on how the molecular clock drives rhythmic gene expression and, consequently, the rhythmicity of biological functions. We started our investigation by following up on the discovery made by Jerome during his post-doc in Michael Rosbash lab that CLOCK:BMAL1 mediates the removal of nucleosomes when it binds DNA, and that this potentiates the recruitment of additional TFs at its enhancers (Menet et al., Genes Dev, 2014). Our results, which we published recently (Trott and Menet, PLoS Genetics, 2018), show that:
(1) CLOCK:BMAL1 rhythmic DNA binding is not sufficient to generate transcriptionally active enhancers/promoters.
(2) the activity of CLOCK:BMAL1 enhancers relies on the activity of transcription factors recruited at nearby binding sites.
(3) the rhythmic expression of many CLOCK:BMAL1 target genes can be remodeled by changes in the environment (e.g., fasting) in spite of any changes of Clock and Bmal1 rhythmic expression.
Altogether, these data suggest that CLOCK:BMAL1 promotes a transcriptionally permissive chromatin landscape that primes its target genes for transcription activation rather than directly activating transcription. They also provide a framework to explain how environmental or pathological conditions can reprogram the rhythmic expression of clock-controlled genes without affecting much of the molecular clock oscillation.
We are currently extending these findings by:
(1) determining how CLOCK:BMAL1 mediates the removal of nucleosomes at its binding sites. Does it require chromatin remodelers, or is nucleosome breathing sufficient? Does CLOCK:BMAL1 facilitates post-transcriptional modifications on histones to disengage nucleosomes from DNA?
(2) determining the modalities of the cooperation between CLOCK:BMAL1 and other transcription factor DNA binding (Fig. 2). Does CLOCK:BMAL1 facilitate the binding of all transcription factors, or only some specific ones? Is the spacing between CLOCK:BMAL1 binding motifs and other transcription factor motifs important? Can other transcription factors modulate CLOCK:BMAL1 DNA binding?

Figure 2: Cooperation between CLOCK:BMAL1 and other transcription factors at CLOCK:BMAL1 enhancers
Adapted from Beytebiere et al., Transcription, 2019.
We further characterized how CLOCK:BMAL1 regulates rhythmic transcription in another study that interrogated how the molecular clock generates tissue-specific rhythms despite relying on identical clock genes in every cell (Beytebiere et al., Genes Dev, 2019). By identifying the genome-wide BMAL1 binding sites in three mouse tissues, we showed that BMAL1 DNA binding is largely tissue-specific because of differences in chromatin accessibility between tissues, and co-binding with tissue-specific transcription factors. We also discovered that BMAL1’s ability to drive tissue-specific rhythmic transcription is not only associated with the activity of BMAL1-bound enhancers (see above), but also with the activity of neighboring enhancers. We substantiated this finding by analyzing physical interactions between BMAL1 enhancers and other cis-regulatory regions in the mouse liver using a technique called ChIA-PET. Our results revealed that rhythmic BMAL1 target gene expression coincides with rhythmic chromatin interactions between BMAL1 enhancers and other cis-regulatory regions, strongly suggesting that BMAL1 target gene transcription depends on BMAL1’s ability to rhythmically regulate a network of enhancers (Fig. 3).
We are extending these findings by:
(1) determining if rhythmic interactions between CLOCK:BMAL1 enhancers and other enhancers are necessary and sufficient for driving rhythmic transcription.
(2) determining if rhythmic interactions between CLOCK:BMAL1 enhancers and other enhancers regulate the activity of other enhancers (e.g., rhythms of H3K27ac modification, RNA polymerase II recruitment, and enhancer RNA expression)
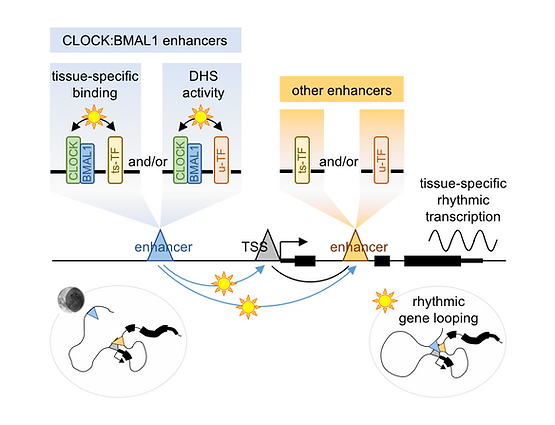
Figure 3: Role of rhythmic chromatin interactions between CLOCK:BMAL1 enhancers and other enhancers in the regulation of rhythmic transcription of CLOCK:BMAL1 target genes.
DHS: DNase I hypersensitive sites (=enhancers and promoters); ts-TF: tissue-specific transcription factor; u-TF: ubiquitously expressed transcription factor; TSS: transcription start site.
From Beytebiere et al., Transcription, 2019.
2. Role of rhythmic food intake in the genome-wide regulation of rhythmic gene expression
Discovery in the early 2000’s that cells cultured in vitro display rhythmic expression of core clock genes led to the notion that rhythmic gene expression is largely driven cell-autonomously by the molecular clock present in every cell, and that the vast majority of transcriptional rhythms in a given tissue depend on an intact local clock (e.g., liver transcriptional rhythms depend on an intact liver clock). However, recent evidence suggest that the daily rhythm of food intake (RFI) may be able to bypass the hepatic clock to initiate rhythmic transcription in mouse liver. We set out to test this possibility by determining the relative contribution of the liver clock vs.RFI in the regulation of rhythmic gene expression (Greenwell et al., Cell Reports, 2019). To this end, we designed a feeding apparatus (see details here) that enables the long-term manipulation of food intake. Specifically, we fed mice arrhythmically across the 24-hour day (access to 1/8th of the daily food intake every 3 hours), and compared gene expression in the liver of wild-type mice fed only at night, ad libitum, or arrhythmically. We found that > 70% of the cycling mouse liver transcriptome loses rhythmicity under arrhythmic feeding (Fig. 4). Surprisingly, this was not due to dysfunction of the liver clock since core clock genes in the liver continued to exhibit normal oscillations in arrhythmically-fed mice. Furthermore, we showed that feeding clock-deficient Bmal1-/- mice only at night restored rhythmic expression of most genes that lost rhythmicity in arrhythmically-fed wild-type mice. These findings demonstrated that 70% of the mouse cycling transcriptome in the liver relies on rhythmic systemic signals driven by RFI rather than on an intact hepatic clock. Interestingly, inspection of genes regulated by RFI showed that RFI manipulation alters the timing of key signaling (e.g., mTOR, Erk) and metabolic (e.g., glycogenesis, lipogenesis) pathways without altering the hepatic clock oscillations. Taken together, our data thus revealed that rhythmic systemic signals can initiate 24-hour rhythms in gene expression in the liver independently of the hepatic molecular clock.
These results have major implications about the function of the circadian system in healthy and diseased states. Specifically, they support the idea that circadian disorders originate from desynchronization between the phase of the cell-autonomous peripheral clocks and the phase of rhythmic systemic signals. For example, physical activity at night (which is prevalent in shift-workers) may disrupt the daily oscillations of food intake and body temperature without affecting core clock gene oscillations. This would disrupt coordination between the thousands of rhythmically expressed transcripts in many tissues and, ultimately, lead to a wide range of diseases.
We are currently extending these findings by:
(1) determining how rhythmic food intake regulates rhythmic gene expression. Are some RFI-driven signaling pathways more particularly involved in the regulation of rhythmic gene expression? Does RFI rhythmically regulate the activity of specific metabolic transcription factors to drive rhythmic gene expression?
(2) determining if our finding that RFI regulates rhythmic gene expression in mouse liver extends to other tissues. Are these effects of RFI specific to the liver? To tissues in the gastro-intestinal tract? Are they observed in the brain?

Figure 4: Rhythmic food intake regulates a large fraction of the mouse liver cycling transcriptome without involving the molecular circadian clock.
Figure from Greenwell et al., Cell Reports, 2019.
3. Isoform-specific regulation of rhythmic gene expression by alternative polyadenylation
Cycling transcriptomes have been characterized in many species and tissues, and revealed that 50% of mammalian genomes are rhythmically expressed somewhere in the body. These analyses were mostly carried out using conventional RNA-Seq datasets, and gene expression was reported after concatenating signals from different transcript isoforms. Yet, it remains possible that the different isoforms being transcribed from a given gene may exhibit differences in rhythmic expression, e.g., some isoforms could be rhythmically expressed while others would be arrhythmic across the 24-hour day. We sought to test this possibility by characterizing the rhythmic expression of distinct isoforms generated by alternative polyadenylation. Alternative polyadenylation (APA) is a mechanism that generates transcript isoforms with different 3’ ends, and which therefore affects 3’ UTR length and/or protein sequence (Fig. 5A). The length of the 3’ UTR impacts RNA stability, mRNA subcellular localization, translation efficiency, and protein function, and defects in APA have been associated with diseases including cancer where many 3’ UTRs are shortened.

Figure 5: Role of alternative polyadenylation in the regulation of rhythmic isoform expression.
A: Diagram illustrating how gene transcription can lead to the expression of multiple APA isoforms.
B: Left: IGV browser view of Col18a1 expression across the 24-hour day. Signal for each time point corresponds to the average of 6 biological replicates. Arrows indicate the different APA isoforms, and the day:night cycle is represented by the white and black bars, respectively. Right: expression of Col18a1 at the gene and APA isoform levels. R = rhythmic expression; AR = arrhythmic expression. The sum of all PAS signal does not exactly match gene signal because of the normalization procedure.

Figure 6: Rhythmic gene expression is APA-isoform specific.
Heatmaps representing the arrhythmically expressed APA isoforms located in a gene containing an APA transcript being rhythmic in NR-fed mice only (RA group). APA isoforms are ordered based on the phase of the rhythmic APA transcript located in the same gene. Only genes showing differential rhythmicity (DODR, p<0.05) were considered. Each heatmap column represent a single liver sample for AR- and NR-fed mice (n=18 total per condition; 6 timepoints, n=3 per time point).
By performing 3’ end RNA-Seq in mouse liver collected across the 24-hour day we found that rhythmic gene expression is largely APA isoform-specific (Fig. 5B), and that many genes characterized as arrhythmically expressed harbor rhythmic APA isoforms (Greenwell et al., Biorxiv, 2020). By analyzing APA isoform expression in single mouse liver cells, we showed that differences in isoform expression are not primarily due to APA isoforms being expressed in different cell subtypes, supporting that the regulation of differential APA isoform expression is mostly allelic. Based on these results, we next compared APA isoform expression between total RNA and nuclear RNA, and found that post-transcriptional events significantly contribute to differences in rhythmicity between APA isoforms. Because co-transcriptional loading of RNA binding proteins on nascent transcript has been proposed to regulate the expression of specific APA isoforms, we also examined if changes in transcription factor activity can alter the expression of specific APA isoforms. Remarkably, our results indicate that transcription factors regulate gene expression in a largely APA isoform-specific manner, i.e., changes in their activity only affect the expression of specific APA isoforms rather than all isoforms. Finally, our investigation of polyadenylation site usage across the day and night also revealed that hundreds of mouse liver genes exhibit a rhythm in 3’ UTR length, with shorter 3’ UTR’s occurring in anticipation of the mouse active phase.
We believe that these findings are important not only in the circadian field, but also more generally in the field of transcriptional regulation of gene expression. Given the recent demonstration that 3’ UTR length regulates protein sub-cellular localization, our results provide the groundwork for determining if proteins can be rhythmically expressed in some subcellular compartments, while being arrhythmically expressed in others. Arguably more importantly, we believe that our data shed new light on the mechanisms underlying 3’ UTR shortening in cancer cells. While current models focus on the role of RNA binding proteins in shifting polyadenylation sites usage from distal to proximal sites, our findings suggest that increased activity of oncogenic transcription factors in cancer cells may promote the expression of transcripts with shorter 3’ UTRs without altering the expression of APA isoforms with longer 3’ UTRs. Moreover, our finding that hundreds of genes exhibit a rhythm in 3’ UTR length with shorter UTRs at dusk in anticipation of the mouse active phase strongly suggests that regulation of 3’ UTR length contributes to how cells regulate their metabolic activity across the 24-hour day. Finally, our data also highlight some of the limitations of conventional RNA-Seq technology for the characterization of differential gene expression, and imply that the use of 3’ end RNA-Seq in combination with conventional RNA-Seq better depicts differences in gene expression between experimental conditions.
We are currently extending our findings by:
(1) determining if APA isoform-specific rhythms lead to proteins carrying out rhythmic functions in some sub-cellular locations while being arrhythmic in others. For example, can COL18A1 be rhythmically expressed in specific location (e.g., extra-cellular matrix) while arrhythmic in other locations (Fig. 5B)?
(2) determining how transcription factors regulate the expression of specific APA isoforms. Is the co-transcriptional loading of RNA binding proteins to nascent transcript critical?